Abstract
Dietary fibres can exert beneficial anti-inflammatory effects through microbially fermented short-chain fatty acid metabolites1,2, although the immunoregulatory roles of most fibre diets and their microbiota-derived metabolites remain poorly defined. Here, using microbial sequencing and untargeted metabolomics, we show that a diet of inulin fibre alters the composition of the mouse microbiota and the levels of microbiota-derived metabolites, notably bile acids. This metabolomic shift is associated with type 2 inflammation in the intestine and lungs, characterized by IL-33 production, activation of group 2 innate lymphoid cells and eosinophilia. Delivery of cholic acid mimics inulin-induced type 2 inflammation, whereas deletion of the bile acid receptor farnesoid X receptor diminishes the effects of inulin. The effects of inulin are microbiota dependent and were reproduced in mice colonized with human-derived microbiota. Furthermore, genetic deletion of a bile-acid-metabolizing enzyme in one bacterial species abolishes the ability of inulin to trigger type 2 inflammation. Finally, we demonstrate that inulin enhances allergen- and helminth-induced type 2 inflammation. Taken together, these data reveal that dietary inulin fibre triggers microbiota-derived cholic acid and type 2 inflammation at barrier surfaces with implications for understanding the pathophysiology of allergic inflammation, tissue protection and host defence.
This is a preview of subscription content, access via your institution
Access options
Subscribe to Journal
Get full journal access for 1 year
199,00 €
only 3,90 € per issue
Tax calculation will be finalised during checkout.
Buy article
Get time limited or full article access on ReadCube.
$32.00
All prices are NET prices.
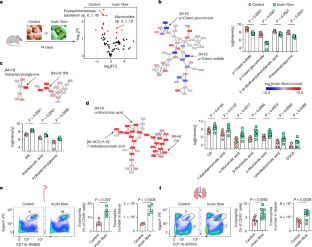
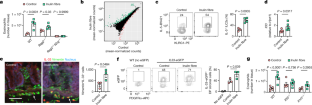
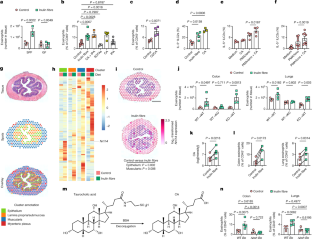
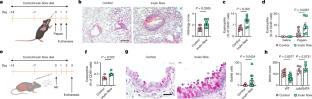
Data availability
All data necessary to understand and evaluate the conclusions of this paper are provided in the Article and its Source Data and Supplementary Information. The 16S rRNA-seq data are available at the NCBI Sequence Read Archive under accession number BioProject PRJNA761331. The RNA-seq and spatial transcriptomic data are available at Gene Expression Omnibus (GEO) under accession numbers GSE183443 and GSE183696, respectively. The mouse genome data (NCBI GRCm38/mm10) used for the alignment of RNA-seq data are available under accession number BioProject PRJNA20689. The MS1 and MS2 data for mouse samples analysed in this study are available at the GNPS website (https://massive.ucsd.edu) under MassIVE ID number MSV000086890. Source data are provided with this paper.
References
-
Brestoff, J. R. & Artis, D. Commensal bacteria at the interface of host metabolism and the immune system. Nat. Immunol. 14, 676–684 (2013).
-
Rooks, M. G. & Garrett, W. S. Gut microbiota, metabolites and host immunity. Nat. Rev. Immunol. 16, 341–352 (2016).
-
Robertson, R. C., Manges, A. R., Finlay, B. B. & Prendergast, A. J. The human microbiome and child growth—first 1000 days and beyond. Trends Microbiol. 27, 131–147 (2019).
-
Fan, Y. & Pedersen, O. Gut microbiota in human metabolic health and disease. Nat. Rev. Microbiol. 19, 55–71 (2021).
-
Belkaid, Y. & Hand, T. W. Role of the microbiota in immunity and inflammation. Cell 157, 121–141 (2014).
-
Blander, J. M., Longman, R. S., Iliev, I. D., Sonnenberg, G. F. & Artis, D. Regulation of inflammation by microbiota interactions with the host. Nat. Immunol. 18, 851–860 (2017).
-
Morais, L. H., Schreiber, H. L. T. & Mazmanian, S. K. The gut microbiota–brain axis in behaviour and brain disorders. Nat. Rev. Microbiol. 19, 241–255 (2020).
-
Chu, C. et al. The microbiota regulate neuronal function and fear extinction learning. Nature 574, 543–548 (2019).
-
Quinn, R. A. et al. Global chemical effects of the microbiome include new bile-acid conjugations. Nature 579, 123–129 (2020).
-
Wikoff, W. R. et al. Metabolomics analysis reveals large effects of gut microflora on mammalian blood metabolites. Proc. Natl Acad. Sci. USA 106, 3698–3703 (2009).
-
Maslowski, K. M. et al. Regulation of inflammatory responses by gut microbiota and chemoattractant receptor GPR43. Nature 461, 1282–1286 (2009).
-
Arpaia, N. et al. Metabolites produced by commensal bacteria promote peripheral regulatory T-cell generation. Nature 504, 451–455 (2013).
-
Furusawa, Y. et al. Commensal microbe-derived butyrate induces the differentiation of colonic regulatory T cells. Nature 504, 446–450 (2013).
-
Smith, P. M. et al. The microbial metabolites, short-chain fatty acids, regulate colonic Treg cell homeostasis. Science 341, 569–573 (2013).
-
Vinelli, V. et al. Effects of dietary fibers on short-chain fatty acids and gut microbiota composition in healthy adults: a systematic review. Nutrients 14, 2559 (2022).
-
Chambers, E. S. et al. Dietary supplementation with inulin-propionate ester or inulin improves insulin sensitivity in adults with overweight and obesity with distinct effects on the gut microbiota, plasma metabolome and systemic inflammatory responses: a randomised cross-over trial. Gut 68, 1430–1438 (2019).
-
De Filippo, C. et al. Impact of diet in shaping gut microbiota revealed by a comparative study in children from Europe and rural Africa. Proc. Natl Acad. Sci. USA 107, 14691–14696 (2010).
-
Schnorr, S. L. et al. Gut microbiome of the Hadza hunter-gatherers. Nat. Commun. 5, 3654 (2014).
-
Thakare, R., Alamoudi, J. A., Gautam, N., Rodrigues, A. D. & Alnouti, Y. Species differences in bile acids I. Plasma and urine bile acid composition. J. Appl. Toxicol. 38, 1323–1335 (2018).
-
Wahlstrom, A., Sayin, S. I., Marschall, H. U. & Backhed, F. Intestinal crosstalk between bile acids and microbiota and its impact on host metabolism. Cell Metab. 24, 41–50 (2016).
-
Klose, C. S. & Artis, D. Innate lymphoid cells as regulators of immunity, inflammation and tissue homeostasis. Nat. Immunol. 17, 765–774 (2016).
-
Saenz, S. A. et al. IL25 elicits a multipotent progenitor cell population that promotes TH2 cytokine responses. Nature 464, 1362–1366 (2010).
-
Klose, C. S. N. & Artis, D. Innate lymphoid cells control signaling circuits to regulate tissue-specific immunity. Cell Res. 30, 475–491 (2020).
-
Klose, C. S. N. et al. The neuropeptide neuromedin U stimulates innate lymphoid cells and type 2 inflammation. Nature 549, 282–286 (2017).
-
Brestoff, J. R. et al. Group 2 innate lymphoid cells promote beiging of white adipose tissue and limit obesity. Nature 519, 242–246 (2015).
-
Nussbaum, J. C. et al. Type 2 innate lymphoid cells control eosinophil homeostasis. Nature 502, 245–248 (2013).
-
Flamar, A. L. et al. Interleukin-33 induces the enzyme tryptophan hydroxylase 1 to promote inflammatory group 2 innate lymphoid cell-mediated immunity. Immunity 52, 606–619 (2020).
-
Halim, T. Y. et al. Group 2 innate lymphoid cells are critical for the initiation of adaptive T helper 2 cell-mediated allergic lung inflammation. Immunity 40, 425–435 (2014).
-
Dahlgren, M. W. et al. Adventitial stromal cells define group 2 innate lymphoid cell tissue niches. Immunity 50, 707–722 (2019).
-
Mahapatro, M. et al. Programming of intestinal epithelial differentiation by IL-33 derived from pericryptal fibroblasts in response to systemic infection. Cell Rep. 15, 1743–1756 (2016).
-
Mahlakoiv, T. et al. Stromal cells maintain immune cell homeostasis in adipose tissue via production of interleukin-33. Sci. Immunol. 4, eaax0416 (2019).
-
Thomas, C., Pellicciari, R., Pruzanski, M., Auwerx, J. & Schoonjans, K. Targeting bile-acid signalling for metabolic diseases. Nat. Rev. Drug Discov. 7, 678–693 (2008).
-
Campbell, C. et al. Bacterial metabolism of bile acids promotes generation of peripheral regulatory T cells. Nature 581, 475–479 (2020).
-
Hang, S. et al. Bile acid metabolites control TH17 and Treg cell differentiation. Nature 576, 143–148 (2019).
-
Song, X. et al. Microbial bile acid metabolites modulate gut RORγ+ regulatory T cell homeostasis. Nature 577, 410–415 (2020).
-
Stahl, P. L. et al. Visualization and analysis of gene expression in tissue sections by spatial transcriptomics. Science 353, 78–82 (2016).
-
Ridlon, J. M., Kang, D. J. & Hylemon, P. B. Bile salt biotransformations by human intestinal bacteria. J. Lipid Res. 47, 241–259 (2006).
-
Singh, V. et al. Dysregulated microbial fermentation of soluble fiber induces cholestatic liver cancer. Cell 175, 679–694 (2018).
-
Qin, J. et al. A human gut microbial gene catalogue established by metagenomic sequencing. Nature 464, 59–65 (2010).
-
Yao, L. et al. A selective gut bacterial bile salt hydrolase alters host metabolism. eLife 7, e37182 (2018).
-
Cruickshank-Quinn, C. I. et al. Metabolomics and transcriptomics pathway approach reveals outcome-specific perturbations in COPD. Sci. Rep. 8, 17132 (2018).
-
Kim, D. J. et al. Metabolic fingerprinting uncovers the distinction between the phenotypes of tuberculosis associated COPD and smoking-induced COPD. Front. Med. 8, 619077 (2021).
-
Novey, H. S., Marchioli, L. E., Sokol, W. N. & Wells, I. D. Papain-induced asthma–physiological and immunological features. J. Allergy Clin. Immunol. 63, 98–103 (1979).
-
Lajoie, S. et al. Complement-mediated regulation of the IL-17A axis is a central genetic determinant of the severity of experimental allergic asthma. Nat. Immunol. 11, 928–935 (2010).
-
Camberis, M., Le Gros, G. & Urban, J.Jr. Animal model of Nippostrongylus brasiliensis and Heligmosomoides polygyrus. Curr. Protoc. Immunol. 55, 19.12.1–19.12.27 (2003).
-
Janssen, A. W. F. et al. Modulation of the gut microbiota impacts nonalcoholic fatty liver disease: a potential role for bile acids. J. Lipid Res. 58, 1399–1416 (2017).
-
McLoughlin, R. et al. Soluble fibre supplementation with and without a probiotic in adults with asthma: a 7-day randomised, double blind, three way cross-over trial. EBioMedicine 46, 473–485 (2019).
-
Karpievitch, Y. V., Dabney, A. R. & Smith, R. D. Normalization and missing value imputation for label-free LC-MS analysis. BMC Bioinform. 13, S5 (2012).
-
Han, H. et al. IL-33 promotes gastrointestinal allergy in a TSLP-independent manner. Mucosal Immunol. 11, 394–403 (2018).
-
Sinal, C. J. et al. Targeted disruption of the nuclear receptor FXR/BAR impairs bile acid and lipid homeostasis. Cell 102, 731–744 (2000).
-
Yu, C. et al. Targeted deletion of a high-affinity GATA-binding site in the GATA-1 promoter leads to selective loss of the eosinophil lineage in vivo. J. Exp. Med. 195, 1387–1395 (2002).
-
Ameri, A. H. et al. IL-33/regulatory T cell axis triggers the development of a tumor-promoting immune environment in chronic inflammation. Proc. Natl Acad. Sci. USA 116, 2646–2651 (2019).
-
Hsu, C. L., Neilsen, C. V. & Bryce, P. J. IL-33 is produced by mast cells and regulates IgE-dependent inflammation. PLoS ONE 5, e11944 (2010).
-
Poeggeler, B. et al. Indole-3-propionate: a potent hydroxyl radical scavenger in rat brain. Brain Res. 815, 382–388 (1999).
-
Rothhammer, V. et al. Type I interferons and microbial metabolites of tryptophan modulate astrocyte activity and central nervous system inflammation via the aryl hydrocarbon receptor. Nat. Med. 22, 586–597 (2016).
-
Friedrich, M. et al. IL-1-driven stromal-neutrophil interactions define a subset of patients with inflammatory bowel disease that does not respond to therapies. Nat. Med. 27, 1970–1981 (2021).
-
Goc, J. et al. Dysregulation of ILC3s unleashes progression and immunotherapy resistance in colon cancer. Cell 184, 5015–5030 (2021).
-
Curtis, J. L., Byrd, P. K., Warnock, M. L. & Kaltreider, H. B. Requirement of CD4-positive T cells for cellular recruitment to the lungs of mice in response to a particulate intratracheal antigen. J. Clin. Invest. 88, 1244–1254 (1991).
-
Cole, J. R. et al. Ribosomal Database Project: data and tools for high throughput rRNA analysis. Nucleic Acids Res. 42, D633–D642 (2014).
-
McMurdie, P. J. & Holmes, S. phyloseq: an R package for reproducible interactive analysis and graphics of microbiome census data. PLoS ONE 8, e61217 (2013).
-
Langmead, B. & Salzberg, S. L. Fast gapped-read alignment with Bowtie 2. Nat. Methods 9, 357–359 (2012).
-
Dodt, M., Roehr, J. T., Ahmed, R. & Dieterich, C. FLEXBAR—flexible barcode and adapter processing for next-generation sequencing platforms. Biology 1, 895–905 (2012).
-
Dobin, A. et al. STAR: ultrafast universal RNA-seq aligner. Bioinformatics 29, 15–21 (2013).
-
Liao, Y., Smyth, G. K. & Shi, W. The R package Rsubread is easier, faster, cheaper and better for alignment and quantification of RNA sequencing reads. Nucleic Acids Res. 47, e47 (2019).
-
Love, M. I., Huber, W. & Anders, S. Moderated estimation of fold change and dispersion for RNA-seq data with DESeq2. Genome Biol. 15, 550 (2014).
-
Yu, G., Wang, L. G., Han, Y. & He, Q. Y. clusterProfiler: an R package for comparing biological themes among gene clusters. OMICS 16, 284–287 (2012).
-
Benjamini, Y. & Hochberg, Y. Controlling the false discovery rate: a practical and powerful approach to multiple testing. J. R. Stat. Soc. B 57, 289–300 (1995).
-
Stuart, T. et al. Comprehensive integration of single-cell data. Cell 177, 1888–1902 (2019).
-
Szklarczyk, D. et al. STRING v11: protein–protein association networks with increased coverage, supporting functional discovery in genome-wide experimental datasets. Nucleic Acids Res. 47, D607–D613 (2019).
-
Helf, M. J., Fox, B. W., Artyukhin, A. B., Zhang, Y. K. & Schroeder, F. C. Comparative metabolomics with Metaboseek reveals functions of a conserved fat metabolism pathway in C. elegans. Nat. Commun. 13, 782 (2022).
-
Tautenhahn, R., Bottcher, C. & Neumann, S. Highly sensitive feature detection for high resolution LC/MS. BMC Bioinform. 9, 504 (2008).
-
Schymanski, E. L. et al. Identifying small molecules via high resolution mass spectrometry: communicating confidence. Environ. Sci. Technol. 48, 2097–2098 (2014).
-
Carr, K., Whiteley, P. & Shattock, P. Development and reproducibility of a novel high-performance liquid-chromatography monolithic column method for the detection and quantification of trans-indolyl-3-acryloylglycine in human urine. Biomed. Chromatogr. 23, 1108–1115 (2009).
-
Letertre, M. P. M. et al. A targeted ultra performance liquid chromatography—tandem mass spectrometric assay for tyrosine and metabolites in urine and plasma: application to the effects of antibiotics on mice. J. Chromatogr. B 1164, 122511 (2021).
-
Correia, M. S. P., Lin, W., Aria, A. J., Jain, A. & Globisch, D. Rapid preparation of a large sulfated metabolite library for structure validation in human samples. Metabolites 10, 415 (2020).
-
Wang, M. et al. Sharing and community curation of mass spectrometry data with Global Natural Products Social Molecular Networking. Nat. Biotechnol. 34, 828–837 (2016).
-
Shannon, P. et al. Cytoscape: a software environment for integrated models of biomolecular interaction networks. Genome Res. 13, 2498–2504 (2003).
-
Salyers, A. A., Shoemaker, N., Cooper, A., D’Elia, J. & Shipman, J. S. 8 genetic methods for Bacteroides species. Methods Microbiol. 29, 229–249 (1999).
-
Koropatkin, N. M., Martens, E. C., Gordon, J. I. & Smith, T. J. Starch catabolism by a prominent human gut symbiont is directed by the recognition of amylose helices. Structure 16, 1105–1115 (2008).
-
Lu, Y., Yao, D. & Chen, C. 2-Hydrazinoquinoline as a derivatization agent for LC-MS-based metabolomic investigation of diabetic ketoacidosis. Metabolites 3, 993–1010 (2013).
Acknowledgements
We thank the members of the Artis laboratory for discussions and reading the manuscript; A. Alonso and other members of the Epigenomics Core and the Microbiome Core of Weill Cornell Medicine for spatial transcriptomic analyses and RNA-seq; all of the contributing members of the JRI IBD Live Cell Bank consortium, which is supported by the JRI, Jill Roberts Center for IBD, Cure for IBD, the Rosanne H. Silbermann Foundation, the Sanders Family and the Weill Cornell Medicine Division of Pediatric Gastroenterology and Nutrition. The cartoons and illustrations were created using BioRender. The chemical structures were created with ChemDraw. This work was supported by the Crohn’s & Colitis Foundation (851136 to M.A., 901000 to W.Z., 937437 to H.Y.); the Sackler Brain and Spine Institute Research (to C.C.); Thomas C. King Pulmonary Fellowship and Weill Cornell Medicine Fund for the Future (to C.N.P.); the WCM Department of Pediatrics Junior Faculty Pilot Award and The Jill Roberts Center Pilot Award for Research in IBD (to A.M.T.); AGA Research Foundation, WCM-RAPP Initiative, the W. M. Keck Foundation (to C.-J.G.); the Howard Hughes Medical Institute (to F.C.S.); the LEO foundation, CURE for IBD, the Jill Roberts Institute for Research in IBD, the Sanders Family Foundation and Rosanne H. Silbermann Foundation (to D.A.); and the National Institutes of Health (5T32HL134629 to C.N.P., DP2 HD101401-01 to C.-J.G., AI140724 to S.W., KL2 TR002385 to A.F.H., R35 GM131877 to F.C.S., and DK126871, AI151599, AI095466, AI095608, AR070116, AI172027 and DK132244 to D.A.).
Author information
Authors and Affiliations
Consortia
Contributions
M.A. performed most of the experiments and analysed the data. T.H.W. and F.C.S. conducted untargeted metabolomic data generation and analysis, T.-T.L. and C.-J.G. performed bacterial genome editing and additional LC–quadrupole time-of-flight MS. A.F.H. and S.W. performed lung function measurement. H.Y., S.D., W.Z., C.N.P., S.K., A.M.T., C.C., Q.W. and W.-B.J. helped with various experiments with mice. G.G.P. and A.G. performed RNA-seq data analyses. The members of the JRI IBD Live Cell Bank Consortium contributed to human sample acquisition and processing. M.A. and D.A. conceived the project, analysed data and wrote the manuscript with input from all of the authors.
Corresponding authors
Ethics declarations
Competing interests
D.A. has contributed to scientific advisory boards at Pfizer, Takeda, FARE and the KRF. F.C.S. is a cofounder of Ascribe Bioscience and Holoclara. The other authors declare no competing interests.
Peer review
Peer review information
Nature thanks Pieter Dorrestein and the other, anonymous, reviewer(s) for their contribution to the peer review of this work.
Additional information
Publisher’s note Springer Nature remains neutral with regard to jurisdictional claims in published maps and institutional affiliations.
Extended data figures and tables
Extended Data Fig. 1 Changes in gut microbiota by inulin fibre diet.
a–d, Mice were fed the control or inulin fibre diets for two weeks (n = 5 mice). Weighted UniFrac PCoA (a) and taxonomic classification (b) of 16S rRNA in stool pellets were shown. For PCoA plot PERMANOVA: F = 12.6, Df = 1, P = 0.008. ‘uncl_d_’ stands for ‘unclassified_domain_’. ‘uncl_d_Bacteria’ matches exactly to mitochondria or chloroplasts, probably from the food. Relative abundance of families or genera increased (c) or decreased (d) by the inulin fibre diet was shown. Data in a–d are representative of three independent experiments. Data are means ± s.e.m. Statistics were calculated by unpaired two-tailed t-test (c,d).
Extended Data Fig. 2 Changes in systemic metabolome by inulin fibre diet.
a, Chemical structures of major differentially abundant compounds identified in this study. b, Faecal concentration of SCFAs in mice from control and inulin fibre diet groups (n = 5 mice). c, Integration of serum bile acids shown in Fig. 1d (n = 6 mice for control or 7 mice for inulin fibre). Data in b,c are representative of at least two independent experiments. Data are means ± s.e.m. Statistics were calculated by two-way ANOVA with Šidák’s multiple-comparisons test (b) or unpaired two-tailed t-test (c).
Extended Data Fig. 3 MS analyses of various samples.
a, Table depicting detection level and other parameters for the metabolites analysed. b–c, Coinjection plots (b) or MS/MS spectra (c) for the metabolites analysed. d–g, Relative abundance of conjugated bile acids in serum (n = 8 mice for control or 9 mice for inulin fibre) (d), faecal unconjugated (e) and conjugated bile acids (f) (n = 9 mice for control or 12 mice for inulin fibre), and conjugated and unconjugated bile acids in caecal content (n = 3 mice) (g). Data are representative of (g) or combined (d–f) from two independent experiments. Data are means ± sem. Statistics were calculated by unpaired two-tailed t-test (d–g).
Extended Data Fig. 5 Effects of inulin fibre diet on immune cells in various tissues.
a,b, Mice were fed control or inulin fibre diet for two weeks (n = 4 mice). Bar graph shows frequencies of FoxP3+ Treg cells (a) and various immunocytes (b) in the colonic lamina propria of control or inulin fibre diet-fed mice. c, Frequency of colonic eosinophils in mice fed control or inulin fibre diet for the indicated periods of time (n = 3 mice for 0.5-, 1-, or 12-week timepoints, n = 5 mice for 2-week timepoint, and n = 4 mice for 6-week timepoint). d, Percentage of eosinophils in various tissue sites from mice administered control or inulin fibre diet for two weeks. mesLN, mesenteric lymph nodes; SI, small intestine. n = 4 for bone marrow, blood, spleen, mesLN, caecum, SI control or skin inulin fibre, n = 3 for SI inulin fibre or skin control. e,f, Mice were fed chow, control diet, or various high fibre diets, and serum levels of CA (e) were measured after two weeks (n = 3 mice for chow, control, or cellulose fibre, and n = 4 mice for inulin or psyllium fibre). Frequency of eosinophils in the colon and lung (f) of mice fed the indicated diets for two weeks (n = 7 mice (colon) or 4 mice (lung) for chow, n = 6 mice for control or inulin fibre, n = 3 mice for cellulose fibre, and n = 7 mice (colon) or 3 mice (lung) for psyllium fibre). Data are representative of (a–d) or combined (e,f) from two independent experiments. Data are means ± s.e.m. Statistics were calculated by unpaired two-tailed t-test (a), two-way ANOVA with Šidák’s (b,d) or Holm–Šidák’s (c) multiple-comparisons test, or one-way ANOVA with Šidák’s multiple-comparisons test (e,f).
Extended Data Fig. 6 Inulin fibre diet-induced gene and protein expression in various immune and non-immune cells.
a, Mice were fed control or inulin fibre diet for two weeks and ILC2s (CD45+Lin−CD90.2+CD127+KLRG1+) were sorted from colonic lamina propria cells. Heatmap showing level of significance of GO enrichment tests in the colonic ILC2 RNASeq data, as measured by -log10(Pcorrected). Blue, not significant (Pcorrected > 0.01), Red, significant (Pcorrected < 0.01). b,c Gating strategy for bar graphs for IL-5+ cells (including Fig. 2c) (b) and frequency of IL-5-expressing CD4+ T cells (c) in the colonic lamina propria of mice (n = 4 mice). d, Gating strategy for detecting PDGFRα+Sca-1+ (double positive, DP) mesenchymal stromal cells (for bar graphs including Fig. 2f) and double negative (DN) stromal cells in colon and lung tissues. e,f Flow cytometry plots and bar graphs showing IL-33-eGFP expression in epithelial and stromal cell subsets in the colon (e) and lung (f). n = 2 mice (No eGFP) or 3 mice (colon control or inulin fibre) or 4 mice (lung control or inulin fibre). Data in c,e,f, are representative of two independent experiments. Data are means ± s.e.m. Statistics were calculated by unpaired two-tailed t-test (c, f) or one-way ANOVA with Holm–Šidák’s multiple-comparisons test (e).
Extended Data Fig. 7 Effects of bile acid metabolites on immune and non-immune cells.
a, Frequency of RORγt+ subset of Tregs in the colons of mice administered SCFAs in drinking water, or water alone (control) (n = 4 mice). b, Serum levels of CA in mice provided with regular or CA-supplemented drinking water (control n = 6 mice, CA n = 4 mice). c, Frequency of eosinophils in the lungs of indicated groups (control n = 4 mice, inulin fibre n = 2 mice, CA n = 4 mice). d, Serum levels of CDCA in mice administered 6 mM CDCA in drinking water for two weeks (control n = 6 mice, CDCA n = 3 mice). e, Percentage of IL-5+ ILC2s in single cells from the colons of naïve WT mice in vitro stimulation with or without 50 μM CA (n = 4 mice). f, Gating strategy for detection of human ILCs (for bar graphs including Fig. 3f). g, Top, STRING network visualization of the genes upregulated by the inulin fibre diet (FDR < 10%) in the indicated layers of mouse colon determined by spatial transcriptomic analyses as shown in Fig. 3h. Lines represent protein–protein associations. Connected clusters of less than 3 nodes (genes), as well as all disconnected nodes were excluded. Bottom, a table shows selected significantly enriched KEGG pathways for the genes shown in the STRING network. h, Il33 expression in WT or Nr1h4−/− mice fed control or inulin fibre diet for two weeks (n = 4 mice for WT inulin fibre group and 3 mice for other groups). i, Frequency of eosinophils in the colons and lungs of WT and Nr1h4−/− mice on control or inulin fibre diet (n = 3 mice). j, Il33 levels determined by qRT-PCR in sorted stromal cells cultured for 7 days followed by stimulation for 24 h with media control or 50 μM CA with or without 10 μM DY268 (n = 5 mice). k, Number of IL-5-expressing ILC2s in the colon of bone marrow chimeric mice on indicated diets (For WT→WT, n = 3 mice for control or 4 mice for inulin fibre, For WT→Nr1h4−/−(KO) or KO→WT, n = 4 mice). Data in a–e, h–k are representative of two independent experiments. Data are means ± s.e.m. Statistics were calculated by Mann–Whitney U-test (a), unpaired (b,d) or paired (e, j) two-tailed t-test or one-way (c) or two-way (h,i,k) ANOVA with Fisher’s LSD test.
Extended Data Fig. 8 Metabolic and immunological parameters in gnotobiotic mice on inulin fibre diet.
a, Schematic diagram for the FMT experiment with human microbiota. b, Taxonomic classification of 16S rRNA genes in faecal suspension from individual human donors or stool pellets collected from representative recipient mice with corresponding human microbiota on control or inulin fibre diet (n = 4 human donors or recipient mice). c–d, Serum CA (c) and tissue eosinophil (d) levels in the diet-fed recipient mice. Each dot represents one animal, and each colour represents one donor. For serum CA levels, n = 15 control mice or 17 inulin fibre mice. For tissue eosinophil levels, n = 14 control mice or 16 inulin fibre mice. e–h, Representative metabolic and immunological parameters of mice colonized with faecal content from one donor two weeks post initiation of the indicated diets (n = 4 mice for control or 5 mice for inulin fibre). Levels of various unconjugated and conjugated bile acid were measured in serum (e) and faeces (f). Il33 expression was measured in the colon (g) and IL-5+ ILC2s in colon and lung (h) two weeks post initiation of the indicated diets. i–j, Single colonies of WT or Δbsh B. ovatus (Bo) strains were cultured in Mega medium with 100 µM taurocholic acid (TCA) for 72 h and then TCA (i) and CA (j) in 100 μl culture supernatants were quantified, n = 3 independent bacterial colonies. k–q, GF mice were monocolonized with WT or Δbsh Bo and fed control or inulin fibre diet. CA and TCA were measured. For serum, n = 10 mice (WT Bo control or Δbsh Bo inulin fibre) or n = 11 mice (WT Bo inulin fibre or Δbsh Bo control). For faeces, n = 6 mice (WT Bo) or n = 8 mice (Δbsh Bo control) or n = 7 mice (Δbsh Bo inulin fibre), and for caecal content, n = 3 mice (WT Bo) or 4 mice (Δbsh Bo) (k–m). Il33 expression in the colon (n), IL-5+ ILC2s in the lung (o), and faecal CFUs (p) were measured (n = 3 mice for WT Bo, n = 4 mice for Δbsh Bo control, n = 3 mice for Δbsh Bo inulin fibre). Expression levels of bsh gene BO_02350 in faecal samples were also quantified (n = 3 mice) (q). Data are representative of (b–j,m–q) or combined (c,d,k,l) from 2-4 independent experiments. Data are means ± s.e.m. Statistics were calculated by unpaired two-tailed t-test (c–j) or two-way ANOVA with Fisher’s LSD test (k–q). The diagram in a was created using BioRender.
Extended Data Fig. 9 The effects of inulin fibre diet in mice challenged with HDM.
a, Schematic diagram for HDM model of allergic airway inflammation. b–d, Frequency of eosinophils in lung (b) and BALF (c) in naïve (saline) or HDM-challenged mice fed the control or inulin fibre diet (saline n = 2 mice, HDM control diet n = 3 mice for lung or 4 mice for BALF, HDM inulin fibre n = 4 mice). d, Airway hyperresponsiveness in HDM-challenged mice measured as resistance of the respiratory system (Rrs) to increasing doses of methacholine (n = 6 mice). Data are representative of (b–c) or combined (d) from 2 independent experiments. Data are means ± s.e.m. Statistics were calculated by unpaired two-tailed t-test (b–c) or two-way ANOVA with Fisher’s LSD test (d). e,Proposed model. The diagrams in a and e were created using BioRender.
Supplementary information
Supplementary Information
Supplementary Figs. 1 and 2 and Supplementary Tables 1 and 2.
Source data
Rights and permissions
Springer Nature or its licensor (e.g. a society or other partner) holds exclusive rights to this article under a publishing agreement with the author(s) or other rightsholder(s); author self-archiving of the accepted manuscript version of this article is solely governed by the terms of such publishing agreement and applicable law.
About this article
Cite this article
Arifuzzaman, M., Won, T.H., Li, TT. et al. Inulin fibre promotes microbiota-derived bile acids and type 2 inflammation. Nature (2022). https://ift.tt/COW2U6t
-
Received:
-
Accepted:
-
Published:
-
DOI: https://ift.tt/COW2U6t
Comments
By submitting a comment you agree to abide by our Terms and Community Guidelines. If you find something abusive or that does not comply with our terms or guidelines please flag it as inappropriate.
"type" - Google News
November 02, 2022 at 11:17PM
https://ift.tt/eWGlg1U
Inulin fibre promotes microbiota-derived bile acids and type 2 inflammation - Nature.com
"type" - Google News
https://ift.tt/uryiLGw
https://ift.tt/X7Y18Zm
Bagikan Berita Ini
0 Response to "Inulin fibre promotes microbiota-derived bile acids and type 2 inflammation - Nature.com"
Post a Comment